Background: Thyroid function tests (TFTs)
are one of the most frequently performed hormonal tests. Abnormal reports are
commonly encountered even in the absence of thyroid dysfunction. Concomitantly
used medications are often implicated in this effect. Objectives:
Failure to recognize drug-induced alterations in TFTs can result in
misdiagnosis and inappropriate management. This review seeks to increase
awareness of the common challenges and pitfalls encountered when interpreting
drug-induced changes in TFTs. Methods: We did a literature search for
eligible publications and articles published before April 2024 on drug-induced alterations in TFTs. Results: The common mechanisms by which drugs alter TFTs include assay interference and alterations in levels or affinity of thyroid-binding proteins (TBP). High-dose biotin can result in erroneous TFTs by interfering with immunoassays that utilize streptavidin-biotin
immobilizing systems. The TFTs mimic findings seen in thyrotoxicosis after biotin intake. Heparin-induced increase in nonessential fatty acid causes displacement of thyroid hormone from TBP, resulting in an artefactual increase in free hormone levels.
Increases or decreases in thyroid-binding globulin (TBG), the major TBP, cause concomitant changes in total thyroxine and triiodothyronine levels. Estrogen, selective estrogen receptor modulators (SERMs), 5-fluorouracil, mitotane, clofibrate, heroin,
and methadone increase, while androgens, anabolic steroids, glucocorticoids, nicotinic acid, and L-asparaginase decrease TBG. Some medications like salicylates, frusemide, carbamazepine, and phenytoin displace thyroxine from TBP, producing a decline
in the total hormone levels. Conclusion: Awareness about these drug-induced TFT fallacies is essential to avoid improper diagnosis and overtreatment.
Keywords: Thyroid function tests, drug interference, biotin,
heparin, thyroid-binding globulin
Thyroid function test (TFT) is one of the most
commonly performed hormonal evaluations. Abnormalities can be detected in TFT
reports in euthyroid individuals due to various reasons. One such important,
but overlooked cause is the interference from concomitant medications during
testing. Various drugs, from over-the-counter vitamin supplements to
specialized anti-cancer medications, can influence the measurement of
components of TFTs, including thyroid-stimulating hormone (TSH), total
thyroxine (TT4), total triiodothyronine (TT3), free thyroxine (FT4), and free
triiodothyronine (FT3).
This review will focus on drugs that produce
fallacious TFTs, in the absence of any clinically relevant effect on thyroid
physiology. Awareness about drug-induced TFT alterations could avert
misdiagnosis and overtreatment of euthyroid subjects.
We searched PubMed, Scopus,
and Google Scholar for eligible publications and articles published before
April 2024 using the following search strategy. We used one of the following
terms: “thyroid function test”, “thyroid assay”, “thyroid-stimulating
hormone”, “thyroxine”, “tri-iodothyronine”,
“thyroid-binding globulin”, “thyroid-binding proteins” in combination with “drugs”, “medications”, “assay interference”, “biotin”, “heparin”, “oral contraceptive pills”, “selective estrogen receptor modulators”, “estrogen”, “tamoxifen”, “raloxifene”,
“droloxifene”, “fluorouracil”, “clofibrate”, “heroin”, “methadone”,
“mitotane”, “nicotinic acid”, “asparaginase”, “glucocorticoids”,
“androgens”, “anabolic steroids”,
“L-asparaginase”, “salicylates”, “aspirin”, “salsalate”, “nonsteroidal anti-inflammatory drugs”,
“frusemide”, “phenytoin”, and “carbamazepine”. References obtained from
original papers were also scrutinized and included wherever relevant to the
subject of this review.
DRUGS INTERFERING WITH THE ASSESSMENT OF THYROID
FUNCTION TESTS
The principal mechanisms by which drugs can cause
inaccurate thyroid test results include assay interference, changes in levels
of serum thyroid-binding proteins (TBP), particularly thyroid-binding globulin
(TBG), and alterations in the affinity or binding of thyroid hormones to serum
TBP. The medications that commonly affect thyroid test results through these
mechanisms will be discussed in the following sections.
DRUGS CAUSING ASSAY INTERFERENCE
Medications can interfere with the accurate
estimation of the thyroid profile due to interference with components of the
assays used. Biotin and heparin are commonly used medications that cause TFT
abnormalities in euthyroid individuals.
Biotin
Biotin based immunoassays:
Biotin is a water-soluble vitamin belonging to vitamin B family. It is also an
essential component of streptavidin-biotin immobilizing systems (SBIS) used for most immunoassays. This system utilizes the interaction between the fungal protein streptavidin and biotin which is one of the strongest noncovalent interactions
in nature. It remains undisturbed by multiple washing steps in assays1.
Furthermore, biotinylation does not alter the
biological activity or immunologic specificity when bound to any test molecule.
SBIS is widely used in many Food and Drug Administration (FDA) approved
immunoassay systems using fully automated platforms, including Access, DxI, and
DxC (Beckman Coulter, California, US); the Elecsys, Cobas, and Modular
platforms (Roche Diagnostics, Basel, Switzerland); the Isys platform (Immuno
Diagnostic System, East Boldon, United Kingdom); the Ortho Vitros platform
(Ortho Clinical Diagnostics, New Jersey, US); the Dimension Vista, Exl,
Immulite platforms (Siemens Healthineers, Erlangen, Germany), Abbott Architect
i2000® (Abbott Diagnostics, Illinois, United States), and Diasorin
Liaison XL® (DiaSorin, Saluggia, Italy)2.
Type of abnormality: The
presence of excess biotin in test samples causes abnormalities in accordance
with the type of immunoassay. Falsely low readings occur with sandwich
immunoassays (glycoprotein hormones like TSH) and falsely high values in
competitive immunoassays (e.g., triiodothyronine (T3), thyroxine (T4), steroid
hormones, and 25-hydroxyvitamin D)2.
Mechanism of interference with
sandwich immunoassays: When the assay has a “sandwich” design
as employed for estimation of TSH, the test serum is incubated with
biotinylated monoclonal TSH antibodies and radiolabeled monoclonal antibodies.
Immune complex “sandwiches” thus formed are captured by streptavidin-coated
magnetic microparticles. Chemiluminescence produced by application of a voltage
to these magnetic microparticles is directly proportional to the TSH levels in
the test serum. The presence of excess biotin in the serum will saturate the
streptavidin-binding sites and reduce binding of these immune sandwiches to the
solid phase (magnetic microparticles) resulting in low chemiluminescence and
falsely low readings as illustrated in Figure 11,2.
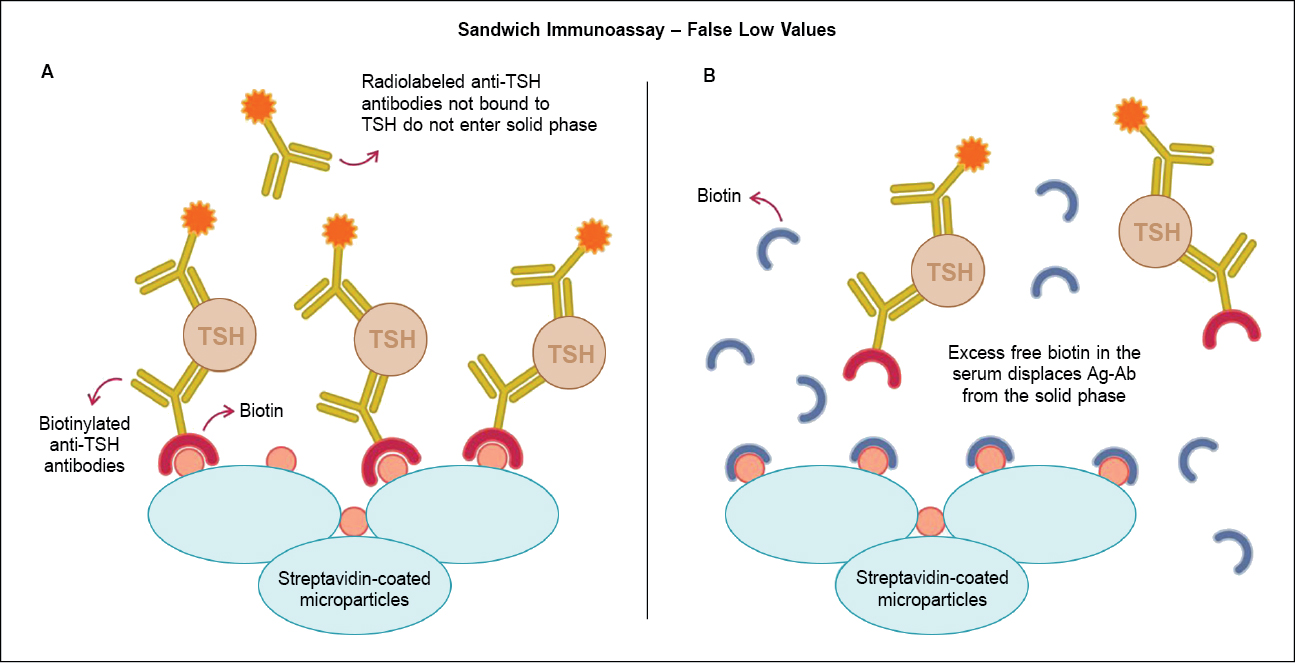
Figure 1. Effect of biotin on sandwich immunoassays utilizing streptavidin-biotin immobilizing systems.
Ag-Ab = Antigen-antibody; TSH = Thyroid-stimulating hormone.
Mechanism of interference with
competitive immunoassays: When a competitive immunoassay is utilized as in
the FT4 assay, the test serum is incubated with biotinylated T4 molecules and
radiolabeled anti-T4 monoclonal antibodies. Biotinylated T4 molecules compete
with the analyte (or T4) in the test serum for binding with the radiolabeled
anti-T4 antibodies. The biotinylated T4 molecules are then immobilized in the
streptavidin-coated microparticles. The chemiluminescence signal generated from
the application of voltage to these magnetic microparticles is inversely
proportional to the levels of FT4. The presence of excess biotin in the test
serum will prevent the complexes of biotinylated T4-radiolabeled antibodies
from binding to the magnetic microparticles. This would result in lower
chemiluminescence, translating to falsely higher levels of T4 in the serum as
depicted in Figure 21,2.

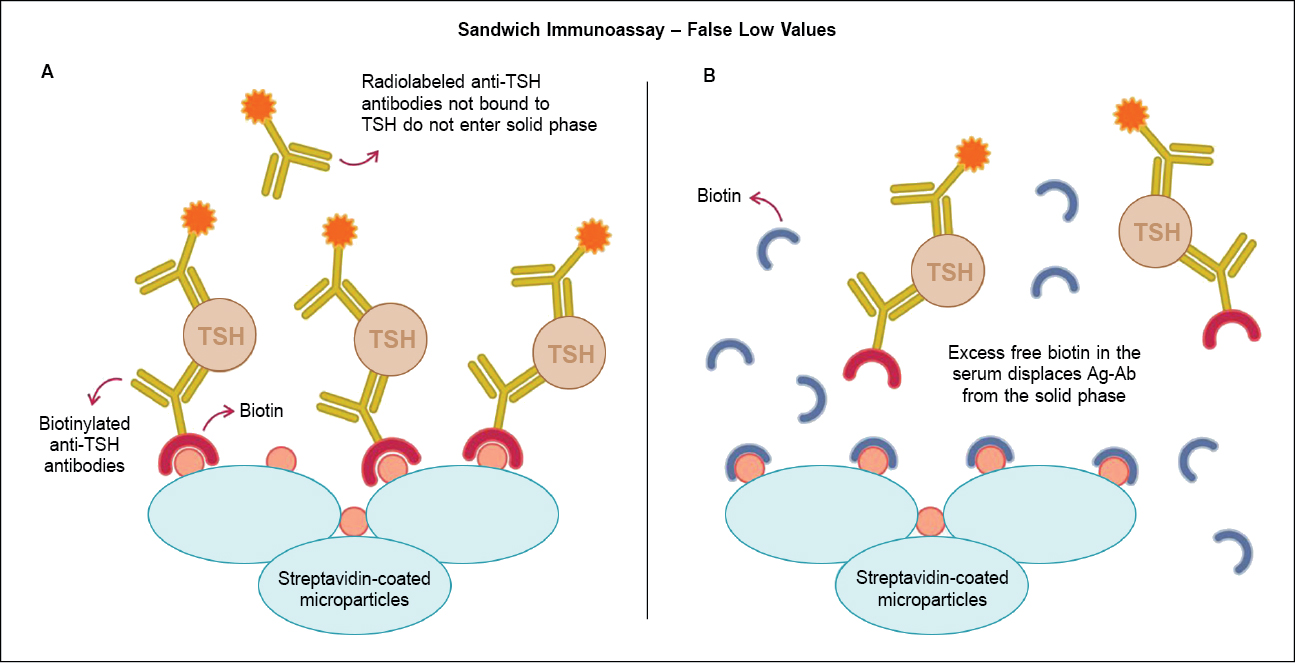
Figure 2. Effect of biotin on competitive immunoassays utilizing streptavidin-biotin immobilizing system.
rT4 = Radiolabeled thyroxine; T4 = Thyroxine.
Biotin dose and assay
interference: The recommended daily intake of biotin ranges from
30 to 70 µg daily. While dietary biotin intake does not generate significant
enough blood levels to interfere with in-vitro diagnostic tests, supraphysiologic
levels achieved through the therapeutic use of biotin as supplements for skin
and hair growth, multiple sclerosis and rare inherited metabolic disorders like
biotinidase deficiency, propionic acidemia, thiamine responsive basal ganglia
disease, holocarboxylase synthase deficiency, and mitochondrial disorders, can
cause problems with these tests3. The minimal dose required and the
degree, duration, and magnitude of the interference is variable and might be
specific to the analyte being tested and the assay characteristics4,5.
The level of interference depends on the serum
concentration attained rather than the dose of biotin consumed, which in turn
is determined by the length of the washout period before retesting6,7,
Grimsey et al evaluated washout periods, required for assays with interference
thresholds ranging from 10 to 100 ng/mL, at biotin dose regimens ranging from 1
mg once daily to 300 mg 4 times daily7. For assays with an in-vitro
interference threshold of >30 ng/mL, biotin doses of up to 5 mg twice daily
or 10 mg once daily, an 8-hour washout period is sufficient to mitigate the
risk. If assays have an in-vitro interference threshold of <30 ng/mL,
or in rare cases of biotin intake of >10 mg/day, sampling should be delayed
for a more extended period (up to 73 h) after the last dose of biotin. Though
the effect of biotin on FT3, FT4, and TSH estimation wanes in hours, anti-TSH
receptor antibodies (TRAbs), which can also be falsely elevated due to assay
interference, may take up to 7 days to normalize2,8-10.
Clinical
correlate: The TFTs could falsely suggest a diagnosis of overt
or subclinical thyrotoxicosis or thyroid hormone resistance, and falsely elevated
TRAbs can further mislead the diagnosis. History of intake of over-the-counter
vitamin supplements containing biotin should be obtained. If an erroneous
report is suspected, retesting should be done in serial dilutions (if using the
same platform) or on another platform, which does not utilize SBIS (Centaur
FT4, Diasorin, Abbott)11,12. Another option would be to repeat the
test, after stopping biotin supplements for a duration which is determined by
the dosage and analyte being assessed. Depletion protocols which involve
pretreatment of the test sample with substances (e.g., streptavidin-coated
particles) that bind biotin can also be used13.
Heparin
TFTs performed in individuals receiving heparin,
can demonstrate fallaciously elevated FT4 and FT3, with normal TSH levels14,15.
Mechanism of interference:
Lipoprotein lipase (LPL), released from vascular endothelium after heparin
exposure, acts on triglycerides, to increase serum levels of nonesterified
fatty acids (NEFA). High NEFA levels inhibit binding of thyroid hormone with
their TBP, producing an increase in the measured free hormone levels14.
There is a demonstrable increase in blood levels of LPL as well as free fatty
acid (FFA), that persists in-vitro, in patients receiving heparin14,15.
Relation to the type of
heparin: The
effect has been reported with the use of unfractionated heparin (UFH) as well
as low molecular weight heparin (LMWH), irrespective of the route or dose.
Standard subcutaneous doses, as well as intravenous doses as small as 0.08
U/kg, can increase the LPL activity of the serum16. It takes
10 hours or more after UFH injection for this effect to remit. Greater
bioavailability and longer duration of action of LMWH might necessitate an
interval of 24 hours after the last dose for this effect to wane17.
Variations depending on assay
and storage: This interference
has been observed with different assays, including direct immunoassays, ultracentrifugation, and equilibrium
dialysis18. Assays that require longer incubation periods (e.g., equilibrium dialysis) are most severely affected. As an extension of the same effect, preanalytical delays due to storage of samples before testing can also worsen
the interference as in-vitro displacement of FT4 and FT3 continues14.
Physiologic variations: The
TFT abnormalities are not seen in all
patients receiving heparin as there are other factors at play, namely serum
levels of triglycerides and albumin. The released LPL requires an adequate concentration of the substrate, i.e., triglycerides
(>180 mg/dL) in the serum to cause
an increase in FFA. Albumin acts as a high-affinity binding protein for FFA;
thus, its serum concentration affects the FFA-induced effects on thyroid
hormone displacement. FT4 levels remain unaltered, till such time that the
molar concentration of FFA is 5 times more than that of albumin, overwhelming
its binding capacity19.
Clinical correlate:
Collecting blood samples after an adequate gap (at least 10 hours with UFH),
immediate processing and testing and correlation with TSH can mitigate the
problem. Estimating TT4 instead of FT4 is more reliable in individuals receiving
heparin and also having hypertriglyceridemia20.
DRUGS AFFECTING THYROXINE-BINDING GLOBULIN
Thyroxine-Binding Globulin Physiology
Thyroxine-binding globulin (TBG) is a high
affinity, low concentration TBP that binds 80% of T3 and 75% of T4. Transthyretin
(TTR) and serum albumin are the other TBPs in the blood. TBG utilizes both
distributive and buffering mechanisms to stabilize the concentration of free
thyroid hormones available to target tissues. This ensures a consistent supply
of thyroid hormones where they are needed in the body21,22.Abnormalities
in the TBG can be inherited or acquired, and drugs are a common etiology for
acquired alteration.
Increase in Thyroxine-Binding Globulin
Estrogen: An elevated TT4 and TT3 level are observed in patients receiving
estrogen, while FT4, FT3, and TSH values remain normal. It causes a
dose-dependent increase in TBG, commencing in 2 weeks of initiation and
reaching a new steady-state within 4 to 8 weeks. At routine doses of ethinyl
estradiol (20-35 µg) or conjugated equine estrogen (CEE) (0.625 mg), there is
an approximate increase of 30% to 50% in TBG and 20% to 35% in TT423-27.
The progesterone component of oral contraceptives (OCs) does not alter this
estrogen-induced effect; however, the androgenic properties of the progesterone
do offset the net effect of the OC on TBG levels. OCs containing antiandrogenic
progesterone like dienogest induce a higher rise in TBG (50%-60% vs. 30%) as
compared to those containing androgenic levonorgestrel28.
Transdermal estrogens do not affect TBG levels despite achieving comparable
serum estrogen levels to oral formulations suggesting the possibility of a
portal threshold for estrogenic stimulation of TBG24,29.
Estrogen increases TBG levels predominantly by prolonging
its half-life, along with a probable slight increase in synthesis. It induces a
post-translational modification of TBG, resulting in molecules which are more
resistant to hepatic degradation, due to the higher terminal sialic acid
content30. The stimulatory effect of estrogen on TBG synthesis, has
been observed in in-vitro studies31,32.
Selective estrogen receptor
modifiers:
Selective estrogen receptor modifiers (SERMs) are molecules that can act as
agonist or antagonist at the estrogen receptors, depending on the target
tissue. Tamoxifen has a weak hepatic estrogen-agonistic effect and can cause a
mild increase in serum TBG concentrations. The increases are lower than those
observed during pregnancy or with estrogen use33-36.
Raloxifene at a daily dose of 60 mg is used for
postmenopausal osteoporosis. Six months of therapy with raloxifene did not significantly affect TBG or TT4 levels37.However, when the same dose of raloxifene was administered for 1 year, it resulted in a small but significant
increase in serum TBG38,39. In postmenopausal women, droloxifene at the dose of 60 mg daily for 6 weeks, increased TBG by 41% from baseline. The increase in TBG with CEE (used as the comparator) was twice that observed with droloxifene.
Although structurally similar to tamoxifen, droloxifene may have a more profound effect on serum TBG. It is unclear whether this is due to greater estrogen agonistic properties on the liver or is dose-related40.
Heroin and methadone: Serum
TBG concentrations are increased in about 25% to 50% of chronic heroin abusers
or those under methadone therapy41-43. In a series of 285 euthyroid
individuals with narcotic addiction, 22% had elevated TT4 and TBG.
Normalization of both parameters after successful stabilization with methadone
therapy44. Inhalational opium use is also associated with an
increase in TT3 levels and TBG as assessed by T3 resin uptake (T3RU)45,46.
T3RU is commonly used to evaluate TBG as there is an inverse association
between T3RU and TBG47.
The mechanisms involved in these changes have not
been clearly understood. The rise in TBG could be related to increased
synthesis or decreased degradation or both. The widely accepted theory is that
of concomitant liver dysfunction, as TBG is synthesized in the liver41,44,48.
In methadone treated addicts, high T3-binding ratio has been demonstrated to
correlate with the degree of hepatic dysfunction directly. The hepatic
dysfunction in these patients is multifactorial with contribution from the
direct effect of opiates on the liver, dietary deficiencies, chronic and
low-grade inflammation due to frequent injections, abuse of other drugs, and OC
use48. Liver dysfunction, however, is unlikely to be the sole
pathogenetic mechanism as these TBG changes are also seen in individuals on
narcotics (8%-25%) with normal liver function44.
Another proposed mechanism is
through inhibition of thyroxine-metabolizing microsomal enzymes in the liver by
morphine (a heroin metabolite), and contaminants (like quinine) and methadone41,42.
Alteration in sex steroids by hepatic dysfunction or direct effects of heroin
or methadone could also influence TBG levels41.
5-Fluorouracil:
5-Fluorouracil has been associated with an increase in TT4 and TT3, but FT4 and
TSH remain unchanged. It is probably due to an increase in TBG levels. There
are not many studies reporting this effect of 5-fluorouracil49.
Mitotane:
Long-term use of mitotane is associated with an increase in the concentration
of TBG and also a smaller increment of sex hormone-binding globulin and
cortisol-binding globulin. The changes occur as early as 1 month after
commencement of therapy and normalize gradually approximately 1 year after
cessation of medications. The exact mechanism is not clear, and presumed to be
related to reduce degradation due to enhanced sialylation or increased
synthesis50.
Interestingly, unlike the other medications which
raise TBG, there is a decrease in TT4 levels by around 38% from the baseline
value50. This is hypothesized to be due to competition of mitotane
for binding sites on TBG or due to alteration in T4-binding characteristics51.
There are also reports of reduced FT4 levels with
prolonged mitotane use, with an inverse correlation between FT4 levels and
serum mitotane concentrations. While the exact reason for this is unknown, in
patients receiving mitotane, high FT3/FT4 ratios have been demonstrated, which
indicate augmented deiodinase activity aiding the conversion of T4 to T352,53.
This is considered as a characteristic compensatory thyroid function change in
hypothyroid conditions54. Changes in FT3 or TSH levels have not been
reported.
Clofibrate:
Clofibrate, a hypolipidemic agent, has been discontinued since 2002, given the
observed excess mortality despite successful cholesterol-lowering55.
Increase in TBG and a small decrease in FT4 and FT3 levels were observed with
this medication56.There was however no relationship
between these changes and the lipid response to clofibrates56,57.
Decrease in Thyroid-Binding Globulin
Androgens and anabolic
steroids: Androgens,
when used as replacement therapy in hypogonadal men or in supraphysiological
doses for anabolic effects in athletes of both sexes, result in decreased TBG,
TT4, and TT3 levels without affecting FT4 and TSH levels58-61. The
rise starts within 1 week, reaching a maximum in 2 to 3 weeks60.
The effect has also been observed with danazol used
in its usual dose of 800 mg daily for treatment of endometriosis62.
Androgen therapy with the nonaromatizable oral fluoxymesterone for metastatic,
hormone-sensitive breast cancer, decreased serum levels of TT4 and TBG in 4
weeks. Normalization occurs within 6 to 12 weeks of therapy cessation. In
hypothyroid women, on levothyroxine supplementation, the decrease in TBG caused
clinical thyrotoxicosis (with elevated FT4 and suppressed TSH), requiring 25%
to 50% dose reduction in thyroid hormone doses. The effect reversed after 8 to
10 weeks of cessation of androgen therapy63. In euthyroid
female-to-male transsexuals, administration of testosterone esters at a dose of
250 mg intramuscular every 2 weeks, resulted in a 14% decrease in TBG but serum
FT4 and TSH levels remained unchanged. The study also reported an increase in
T3/T4 ratio indicating a probable upregulation of deiodinase activity by
testosterone29.
Though the exact mechanism is unclear; it is
believed that androgens cause desialylation of TBG making it susceptible to
degradation63.
The net effect of a particular androgen on TBG
levels depends on its aromatization rate to estrogen60. Adequacy of
liver function also influences this effect, as observed in a study in males
with alcoholic cirrhosis. Oral testosterone treatment resulted in TBG decrease
in alcoholic cirrhotic men with mild liver dysfunction (Child-Turcotte Group A)
and not in those with more advanced liver disease (Child-Turcotte Group B
or C). This could be due to inadequate expression of sex hormone receptors
in the liver, increase in serum estrogen concentration and use of
antiandrogenic medication like spironolactone64. Short-term studies
have suggested a possible shift of T4 binding from TBG to transthyretin during
therapy with norethandrolone due to lower TBG levels65. From a
clinical perspective, androgen therapy may unmask or worsen mild
hyperthyroidism due to reduction of TBG levels66.
Chronic glucocorticoid
therapy: Endogenous
glucocorticoid hypersecretion or exogenous glucocorticoid administration, at a
rate of 2 to 20 times the basal adrenal glucocorticoid secretion, results in
decreased TBG levels67-69. While it can occur with any route of
administration, it depends on the dose and duration of the glucocorticoid
therapy69. It is unclear how glucocorticoids cause this change; it
is probably mediated through a decrease in the hepatic synthesis of TBG perhaps
at a transcriptional level68.
L-asparaginase:
L-asparaginase decrease TBG levels, with a return to normal after drug
withdrawal. The dose-dependent suppression of hepatic synthesis of the
glycoprotein as demonstrated in in-vitro studies of cultured human
hepatoma (HEP G2) cell lines may be responsible70. During the
induction phase of acute lymphoblastic leukemia (ALL) treatment, L-asparaginase
causes a reduction in TBG and TT471-73. It occurs within 2 days to 2
weeks after administration and resolves in 2 to 4 weeks after cessation of
medication. Two cases of transient hyperthyroidism occurring after
L-asparaginase therapy for ALL, have been reported. There was an increase in
FT4 along with TSH suppression accompanied by clinical features of
thyrotoxicosis in both the cases74.
The mechanism involved is presumed to be due to the
general ability of L-asparaginase to suppress protein synthesis in the liver
along with concomitantly suppressing the production of TBG70.
Nicotinic acid:
Nicotinic acid can result in decreased TBG and TT4 levels without affecting FT4
and TSH values75-78.O’Brien
et al proposed nicotinic acid associated hepatitis or subclinical liver
dysfunction, while Drinka et al attributed a direct drug effect as the cause for the change in TBG76,77.Shakir et al ascribed mobilization of fatty acids from the periphery, with subsequent interference to binding of thyroid hormones
to TBG as another mechanism75. The exact mechanism is not clearly understood.
DRUGS ALTERING THE BINDING OF THYROID HORMONE TO
THYROID-BINDING PROTEINS
Displacement of thyroid hormones from TBPs like TBG
and TTR can alter thyroid test results.
Salicylates and Nonsteroidal Anti-inflammatory Drugs
Salicylates and salsalate inhibit binding of T4 to
all three TBPs with maximum effect on TBG79,80. This occurs at doses
of >2 g daily of salicylates and 1.5-3 g daily of salsalate. There is an
initial and transient increase in FT4 levels at drug initiation, which is then
followed by a decrease in TT4 and normalization of FT4 levels as therapeutic
concentrations are maintained81. Salsalate may produce a more
significant decline in T4 levels as compared to salicylate (30%-40% vs.
20%-30%)80,82.
An analysis of different nonsteroidal
anti-inflammatory drugs (NSAIDs) revealed that ibuprofen, naproxen, and
indomethacin did not alter hormone levels, while aspirin caused a decrease in
TT4, TT3, TSH after 1 week probably due to dilutional artefact. Meclofenamate
caused transient fluctuation of hormone levels which normalized in a week. All
subjects remained clinically euthyroid during the 1 week study period, and TSH
remained within normal range83.
Frusemide
Frusemide displaces thyroid hormones from TBG, TTR,
and albumin, causing a transient increase in FT4 and decrease in TT484.
The effect occurs at large intravenous doses of >80 mg and not at usual
therapeutic doses67. The changes depend on the interval between drug
administration and sample collection, occurring 2 to 5 hours after frusemide
doses of 80, 120, or 250 mg85. Other factors involved are the renal
drug clearance rate and serum concentration of albumin, which also binds
frusemide67.
Phenytoin and Carbamazepine
Phenytoin and carbamazepine lower TT4, TT3, FT3,
and FT4 levels without affecting serum TSH levels, in euthyroid individuals86.
These drugs at therapeutic levels displace thyroid hormones from TBP. This is
distinct from the class effect of augmented T4 and T3 metabolism, by induction
of hepatic microsomal P450 enzymes. Low FT4 levels are presumably artefactual,
and is believed to occur due to serum dilution during the estimation process87.
Direct measurement of FT4 by equilibrium dialysis or indirect assessment by
measuring FT4 index can overcome the effect88.
Several medications can lead to abnormal TFT
results in euthyroid individuals, despite no clinically significant impact on
thyroid function itself. This can occur due to assay interference, changes in
TBP levels, or alterations in their affinity for thyroid hormones. Awareness of
these drug-related effects is crucial because while they may not require
medical intervention, they can potentially mislead clinicians.
1.
Diamandis EP, Christopoulos TK. The biotin-(strept)avidin system:
principles and applications in biotechnology. Clin Chem. 1991;37(5):625-36.
2.
Odhaib SA, Mansour AA, Haddad NS. How biotin induces
misleading results in thyroid bioassays: case series. Cureus. 2019;11(5):e4727.
3.
Evans N, Yates J, Tobin J, McGill J, Huynh T. Immunoassay interference secondary to
therapeutic high-dose biotin: a paediatric case report. J Paediatr Child
Health. 2018;54(5):572-5.
4.
Sturgeon CM, Viljoen A. Analytical error and interference in
immunoassay: minimizing risk. Ann Clin Biochem. 2011;48(Pt 5):418-32.
5.
Al-Salameh A, Becquemont
L, Brailly-Tabard S, Aubourg P, Chanson P. A somewhat bizarre case of Graves disease due to
vitamin treatment. J Endocr Soc. 2017;1(5):431-5.
6.
Paketçi A, Köse E, Gürsoy Çalan Ö, Acar S, Teke P,
Demirci F. Serum level of biotin rather than the daily dosage is the main
determinant of interference on thyroid function assays. Horm Res Paediatr.
2019;92(2):92-8.
7.
Grimsey P, Frey N, Bendig G, Zitzler J, Lorenz O, Kasapic D,
et al. Population pharmacokinetics of exogenous biotin and the relationship
between biotin serum levels and in vitro immunoassay interference. Int J
Pharmacokinet. 2017;2(4):247-56.
8.
Kummer S, Hermsen D, Distelmaier F. Biotin treatment mimicking Graves’ disease. N
Engl J Med. 2016;375(7):704-6.
9.
Sharma A, Baumann NA, Shah P. Biotin-induced biochemical Graves disease: a teachable
moment. JAMA Intern Med. 2017;177(4):571-2.
10.
Kwok JS, Chan IH, Chan MH. Biotin interference on TSH and free thyroid hormone
measurement. Pathology. 2012;44(3):278-80.
11.
Bowen R, Benavides R, Colón-Franco JM, Katzman BM, Muthukumar A, Sadrzadeh H, et al.
Best practices in mitigating the risk of biotin interference with laboratory
testing. Clin Biochem. 2019;74:1-11.
12.
Favresse J, Burlacu MC, Maiter D, Gruson D. Interferences with
thyroid function immunoassays: clinical implications and detection algorithm.
Endocr Rev. 2018;39(5):830-50.
13.
Piketty ML, Prie D, Sedel F, Bernard D, Hercend C, Chanson P,
et al. High-dose biotin therapy leading to false biochemical endocrine
profiles: validation of a simple method to overcome biotin interference. Clin
Chem Lab Med. 2017;55(6):817-25.
14.
Laji K, Rhidha B, John R, Lazarus J, Davies JS. Abnormal serum free thyroid hormone
levels due to heparin administration. QJM. 2001;94(9):471-3.
15.
Burch HB. Drug effects on the thyroid. N Engl J Med. 2019;381(8):749-61.
16.
Jaume JC, Mendel CM, Frost PH, Greenspan FS, Laughton CW. Extremely low doses of heparin
release lipase activity into the plasma and can thereby cause artifactual
elevations in the serum-free thyroxine concentration as measured by equilibrium
dialysis. Thyroid. 1996;6(2):79-83.
17.
Stevenson HP, Archbold GP, Johnston P, Young IS, Sheridan B.
Misleading serum free thyroxine results during low molecular weight heparin
treatment. Clin Chem. 1998;44(5):1002-7.
18.
Koulouri O, Moran C, Halsall D, Chatterjee K, Gurnell M.
Pitfalls in the measurement and interpretation of thyroid function tests. Best
Pract Res Clin Endocrinol Metab. 2013;27(6):745-62.
19.
Mendel CM, Frost PH, Cavalieri RR. Effect of free fatty acids
on the concentration of free thyroxine in human serum: the role of albumin. J
Clin Endocrinol Metab. 1986;63(6):1394-9.
20.
Stockigt JR, Lim CF. Medications that distort in vitro tests of
thyroid function, with particular reference to estimates of serum free
thyroxine. Best Pract Res Clin Endocrinol Metab. 2009;23(6):753-67.
21.
Braverman LE, Cooper D. Werner & Ingbar’s The Thyroid: A
Fundamental and Clinical Text. Lippincott Williams & Wilkins; 2012.
22.
Schussler GC. The thyroxine-binding proteins. Thyroid.
2000;10(2):141-9.
23.
Knopp RH, Bergelin RO, Wahl PW, Walden CE, Chapman MB. Clinical chemistry alterations
in pregnancy and oral contraceptive use. Obstet Gynecol. 1985;66(5):682-90.
24.
Steingold KA, Matt DW, DeZiegler D, Sealey JE, Fratkin M,
Reznikov S. Comparison of transdermal to oral estradiol administration on
hormonal and hepatic parameters in women with premature ovarian failure.
J Clin Endocrinol Metab. 1991;73(2):275-80.
25.
Kuhl H, Jung-Hoffmann C, Weber J, Boehm BO. The effect of a biphasic
desogestrel-containing oral contraceptive on carbohydrate metabolism and
various hormonal parameters. Contraception. 1993;47(1):55-68.
26.
Geola FL, Frumar AM, Tataryn IV, Lu KH, Hershman JM, Eggena P, et al. Biological
effects of various doses of conjugated equine estrogens in postmenopausal
women. J Clin Endocrinol Metab. 1980;51(3):620-5.
27.
Ben-Rafael Z, Struass JF, Arendash-Durand B, Mastroianni L,
Flickinger GL. Changes in thyroid function tests and sex hormone binding
globulin associated with treatment by gonadotropin. Fertil Steril.
1987;48(2):318-20.
28.
Wiegratz I, Kutschera E, Lee JH, Moore C, Mellinger U,
Winkler UH, et al. Effect of four different oral contraceptives on various sex
hormones and serum-binding globulins. Contraception. 2003;67(1):25-32.
29.
Bisschop PH, Toorians AW, Endert E, Wiersinga WM, Gooren
LJ, Fliers E. The effects of sex-steroid administration on the
pituitary–thyroid axis in transsexuals. Eur J Endocrinol. 2006;155(1):11-6.
30.
Ain KB, Mori Y, Refetoff S. Reduced clearance rate of thyroxine-binding globulin
(TBG) with increased sialylation: a mechanism for estrogen-induced elevation of
serum TBG concentration. J Clin Endocrinol Metab. 1987;65(4):689-96.
31.
Glinoer D, Gershengorn MC, Dubois A, Robbins J. Stimulation of
thyroxine-binding globulin synthesis by isolated rhesus monkey hepatocytes
after in vivo beta-estradiol administration. Endocrinology. 1977;100(3):807-13.
32.
Glinoer D, McGuire RA, Gershengorn MC, Robbins J, Berman
M. Effects of estrogen on thyroxine-binding globulin metabolism in rhesus
monkeys. Endocrinology. 1977;100(1):9-17.
33.
Gordon D, Beastall GH, McArdle CS, Thomson JA. The effect of tamoxifen therapy on
thyroid function tests. Cancer. 1986;58(7):1422-5.
34.
Kostoglou-Athanassiou I, Ntalles K, Markopoulos C, Athanassiou P, Gogas J,
Proukakis C. Thyroid function in postmenopausal women with breast cancer on
tamoxifen. Eur J Gynaecol Oncol. 1998;19(2):150-4.
35.
Mamby CC, Love RR, Lee KE. Thyroid function test changes with adjuvant tamoxifen
therapy in postmenopausal women with breast cancer. J Clin Oncol.
1995;13(4):854-7.
36.
Anker GB, Lønning PE, Aakvaag A, Lien EA. Thyroid function in postmenopausal breast
cancer patients treated with tamoxifen. Scand J Clin Lab Invest.
1998;58(2):103-7.
37.
Duntas LH, Mantzou E, Koutras DA. Lack of substantial effects
of raloxifene on thyroxine-binding globulin in postmenopausal women: dependency
on thyroid status. Thyroid. 2001;11(8):779-82.
38.
Hsu SH, Cheng WC, Jang MW, Tsai KS. Effects of long-term use of raloxifene, a
selective estrogen receptor modulator, on thyroid function test profiles. Clin
Chem. 2001;47(10):1865-7.
39.
Ceresini G, Morganti S, Rebecchi I, Bertone L, Ceda GP, Bacchi-Modena
A, et al. A one-year follow-up on the effects of raloxifene on thyroid function
in postmenopausal women. Menopause. 2004;11(2):176-9.
40.
Marqusee E, Braverman LE, Lawrence JE, Carroll JS, Seely EW.
The effect of droloxifene and estrogen on thyroid function in postmenopausal women.
J Clin Endocrinol Metab. 2000;85(11):4407-10.
41.
Azizi F, Vagenakis AG, Braverman LE, Ingbar SH. Thyroxine transport and metabolism
in methadone and heroin addicts. Ann Intern Med. 1974;80(2):194-9.
42.
English TN, Ruxton D, Eastman CJ. Abnormalities in thyroid
function associated with chronic therapy with methadone. Clin Chem.
1988;34(11):2202-4.
43.
Bastomsky CH, Dent RR, Tolis G. Elevated serum concentrations of
thyroxine-binding globulin and caeruloplasmin in methadone-maintained patients.
Clin Biochem. 1977;10(3):124-6.
44.
Webster JB, Coupal JJ, Cushman P. Increased serum thyroxine
levels in euthyroid narcotic addicts. J Clin Endocrinol Metab.
1973;37(6):928-34.
45.
Gozashti MH, Mohammadzadeh E, Divsalar K, Shokoohi M. The effect
of opium addiction on thyroid function tests. J Diabetes Metab Disord.
2014;13(1):5.
46.
Shahsavar F, Ghanadi K, Jafarzadeh M, Nasiri B, Forutani S.
Opium addiction decreases T3 uptake amount. Life Sci J. 2013;10(5s):608-10.
47.
Yan Z, Yan H, Ou H. Human thyroxine binding globulin (TBG) promoter directs
efficient and sustaining transgene expression in liver-specific pattern. Gene.
2012;506(2):289-94.
48.
Schussler GC, Stimmel B, Korn F. Increased serum thyroid hormone
binding in narcotic addicts is due to liver disease. Am J Drug Alcohol Abuse.
1980;7(3-4):379-87.
49.
Beex L, Ross A, Smals A, Kloppenborg P. 5-fluorouracil-induced increase of total
serum thyroxine and triiodothyronine. Cancer Treat Rep. 1977;61(7):1291-5.
50.
Van Seters AP, Moolenaar AJ. Mitotane increases the blood
levels of hormone-binding proteins. Acta Endocrinol
(Copenh). 1991;124(5):526-33.
51.
Marshall JS, Tompkins LS. Effect of o,p’-DDD and similar
compounds on thyroxine binding globulin. J Clin Endocrinol Metab.
1968;28(3):386-92.
52.
Daffara F, De Francia S, Reimondo G, Zaggia B, Aroasio E,
Porpilia F, et al. Prospective evaluation of mitotane toxicity in
adrenocortical cancer patients treated adjuvantly. Endocr Relat Cancer.
2008;15(4):1043-53.
53.
Russo M, Scollo C, Pellegriti G, Cotta OR, Squatrito S, Frasca F, et al.
Mitotane treatment in patients with adrenocortical cancer causes central
hypothyroidism. Clin Endocrinol (Oxf). 2016;84(4):614-9.
54.
Bianco AC, Kim BW. Deiodinases: implications of the local
control of thyroid hormone action. J Clin Invest. 2006;116(10):2571-9.
55.
WHO cooperative trial on primary prevention of ischaemic heart
disease with clofibrate to lower serum cholesterol: final mortality follow-up.
Report of the Committee of Principal Investigators. Lancet. 1984;2(8403):600-4.
56.
McKerron CG, Scott RL, Asper SP, Levy RI. Effects of clofibrate
(Atromid S) on the thyroxine-binding capacity of thyroxine-binding globulin and
free thyroxine. J Clin Endocrinol Metab. 1969;29(7):957-61.
57.
Barbosa J, Oliner L. Effect of clofibrate on serum thyroxine
transport and free thyroxine levels. Metabolism. 1969;18(2):141-7.
58.
Deyssig R, Weissel M. Ingestion of androgenic-anabolic
steroids induces mild thyroidal impairment in male body builders. J Clin
Endocrinol Metab. 1993;76(4):1069-71.
59.
Malarkey WB, Strauss RH, Leizman DJ, Liggett M, Demers LM. Endocrine
effects in female weight lifters who self-administer testosterone and anabolic
steroids. Am J Obstet Gynecol. 1991;165(5 Pt 1):1385-90.
60.
Kley HK, Herrmann J, Morgner KD, Krüskemper HL. Effects of testosterone
oenanthate on plasma concentrations of thyroxine, cortisol, testosterone and
hormone binding proteins in patients with hypogonadism. Horm Metab Res.
1973;5(4):271-4.
61.
Small M, Beastall GH, Semple CG, Cowan RA, Forbes CD.
Alteration of hormone levels in normal males given the anabolic steroid stanozolol.
Clin Endocrinol (Oxf). 1984;21(1):49-55.
62.
Graham RL, Gambrell RD. Changes in thyroid function tests
during danazol therapy. Obstet Gynecol. 1980;55(3):395-7.
63.
Arafah BM. Decreased levothyroxine requirement in women with
hypothyroidism during androgen therapy for breast cancer. Ann Intern Med.
1994;121(4):247-51.
64.
Becker U, Gluud C, Bennett P; The Copenhagen Study Group for Liver Diseases. The
effect of oral testosterone on serum TBG levels in alcoholic cirrhotic men.
Liver. 1988;8(4):219-24.
65.
Braverman LE, Ingbar SH. Effects of norethandrolone on the
transport in serum and peripheral turnover of thyroxine. J Clin Endocrinol
Metab. 1967;27(3):389-96.
66.
Tahboub R, Arafah BM. Sex steroids and the thyroid. Best Pract
Res Clin Endocrinol Metab. 2009;23(6):769-80.
67.
Surks MI, Sievert R. Drugs and thyroid function. N Engl J Med. 1995;333(25):1688-94.
68.
Emerson CH, Seiler CM, Alex S, Fang SL, Mori Y, DeVito WJ. Gene
expression and serum thyroxine-binding globulin are regulated by adrenal status
and corticosterone in the rat. Endocrinology. 1993;133(3):1192-6.
69.
Oppenheimer JH, Werner SC. Effect of prednisone on
thyroxine-binding proteins. J Clin Endocrinol Metab. 1966;26(7):715-21.
70.
Bartalena L, Martino E, Antonelli A, Pacchiarotti A, Robbins J,
Pinchera A. Effect of the antileukemic agent L-asparaginase on
thyroxine-binding globulin and albumin synthesis in cultured human hepatoma
(HEP G2) cells. Endocrinology. 1986;119(3):1185-8.
71.
Ferster A, Glinoér D, Van Vliet G, Otten J. Thyroid function
during l-asparaginase therapy in children with acute lymphoblastic leukemia:
difference between induction and late intensification. Am J Pediatr Hematol
Oncol. 1992;14(3):192-6.
72.
Heidemann PH, Stubbe P, Beck W. Transient secondary
hypothyroidism and thyroxine binding globulin deficiency in leukemic children
during polychemotherapy: an effect of L-asparaginase. Eur J Pediatr.
1981;136(3):291-5.
73.
Garnick MB, Larsen PR. Acute deficiency of thyroxine-binding
globulin during L-asparaginase therapy. N Engl J Med. 1979;301(5):252-3.
74.
Fadilah SA, Faridah I, Cheong SK. Transient hyperthyroidism
following L-asparaginase therapy for acute lymphoblastic leukemia. Med J
Malaysia. 2000;55(4):513-5.
75.
Shakir KM, Kroll S, Aprill BS, Drake AJ, Eisold JF. Nicotinic
acid decreases serum thyroid hormone levels while maintaining a euthyroid
state. Mayo Clin Proc. 1995;70(6):556-8.
76.
O’Brien T, Silverberg JD, Nguyen TT. Nicotinic acid-induced
toxicity associated with cytopenia and decreased levels of thyroxine-binding
globulin. Mayo Clin Proc. 1992;67(5):465-8.
77.
Drinka PJ. Alterations in thyroid and hepatic function tests
associated with preparations of sustained-release niacin. Mayo Clin Proc.
1992;67(12):1206.
78.
Cashin-Hemphill L, Spencer CA, Nicoloff JT, Blankenhorn DH, Nessim SA,
Chin HP, et al. Alterations in serum thyroid hormonal indices with
colestipol-niacin therapy. Ann Intern Med. 1987;107(3):324-9.
79.
Wang R, Nelson JC, Wilcox RB. Salsalate and salicylate binding to and their
displacement of thyroxine from thyroxine-binding globulin, transthyretin, and
albumin. Thyroid. 1999;9(4):359-64.
80.
Larsen PR. Salicylate-induced increases in free triiodothyronine
in human serum. Evidence of inhibition of triiodothyronine binding to
thyroxine-binding globulin and thyroxine-binding prealbumin. J Clin
Invest. 1972;51(5):1125-34.
81.
Faber J, Waetjen I, Siersbaek-Nielsen K. Free thyroxine measured in undiluted serum
by dialysis and ultrafiltration: effects of non-thyroidal illness, and an acute
load of salicylate or heparin. Clin Chim Acta Int J Clin Chem. 1993;223(1-2):159-67.
82.
McConnell RJ. Abnormal thyroid function test results in patients
taking salsalate. JAMA. 1992;267(9):1242-3.
83.
Samuels MH, Pillote K, Asher D, Nelson JC. Variable effects of
nonsteroidal antiinflammatory agents on thyroid test results. J Clin Endocrinol
Metab. 2003;88(12):5710-6.
84.
Stockigt JR, Lim CF, Barlow JW, Wynne KN, Mohr VS, Topliss DJ,
et al. Interaction of furosemide with serum thyroxine-binding sites: in vivo
and in vitro studies and comparison with other inhibitors. J Clin Endocrinol Metab.
1985;60(5):1025-31.
85.
Newnham HH, Hamblin PS, Long F, Lim CF, Topliss DJ,
Stockigt JR. Effect of oral frusemide on diagnostic indices of thyroid
function. Clin Endocrinol (Oxf). 1987;26(4):423-31.
86.
Zhang YX, Shen CH, Lai QL, Fang GL, Ming WJ, Lu RY, et al. Effects of antiepileptic
drug on thyroid hormones in patients with epilepsy: a meta-analysis. Seizure.
2016;35:72-9.
87.
Surks MI, DeFesi CR. Normal serum free thyroid hormone concentrations in patients
treated with phenytoin or carbamazepine. A paradox resolved. JAMA.
1996;275(19):1495-8.
88.
Chopra
IJ, Van Herle AJ, Teco GN, Nguyen AH. Serum free thyroxine in thyroidal and
nonthyroidal illnesses: a comparison of measurements by radioimmunoassay,
equilibrium dialysis, and free thyroxine index. J Clin Endocrinol Metab.
1980;51(1):135-43.